3D reconstructions of parasite development and the intracellular niche of the microsporidian pathogen ... - Nature.com
Abstract
Microsporidia are an early-diverging group of fungal pathogens with a wide host range. Several microsporidian species cause opportunistic infections in humans that can be fatal. As obligate intracellular parasites with highly reduced genomes, microsporidia are dependent on host metabolites for successful replication and development. Our knowledge of microsporidian intracellular development remains rudimentary, and our understanding of the intracellular niche occupied by microsporidia has relied on 2D TEM images and light microscopy. Here, we use serial block-face scanning electron microscopy (SBF-SEM) to capture 3D snapshots of the human-infecting species, Encephalitozoon intestinalis, within host cells. We track E. intestinalis development through its life cycle, which allows us to propose a model for how its infection organelle, the polar tube, is assembled de novo in developing spores. 3D reconstructions of parasite-infected cells provide insights into the physical interactions between host cell organelles and parasitophorous vacuoles, which contain the developing parasites. The host cell mitochondrial network is substantially remodeled during E. intestinalis infection, leading to mitochondrial fragmentation. SBF-SEM analysis shows changes in mitochondrial morphology in infected cells, and live-cell imaging provides insights into mitochondrial dynamics during infection. Our data provide insights into parasite development, polar tube assembly, and microsporidia-induced host mitochondria remodeling.
Similar content being viewed by others
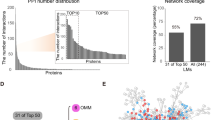
Cross-link assisted spatial proteomics to map sub-organelle proteomes and membrane protein topologies
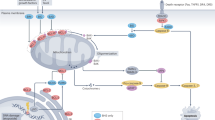
A guide to cell death pathways
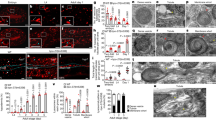
The HEAT repeat protein HPO-27 is a lysosome fission factor
Introduction
Microsporidia, related to fungi, are obligate intracellular pathogens1 that infect a wide range of invertebrate and vertebrate hosts, including humans2,3,4,5,6,7,8,9. In humans, microsporidia most often infect the gastrointestinal tract and cause diarrheal diseases that are self-limiting, but in immunocompromised patients, infections can be fatal5,10,11,12. Microsporidia infect ~5% of the human population13 and have significant impacts on agriculture, aquaculture, and other industries14. Despite their prevalence and global impact, microsporidian biology remains understudied. The species Encephalitozoon intestinalis5 is one of the most common causes of microsporidian infection in humans. Like other microsporidian species, E. intestinalis employs a unique, harpoon-like invasion organelle called the polar tube (PT) to gain entry into a new host cell15,16,17. The PT, many times the length of a microsporidian cell, is coiled up inside the dormant spore. To initiate infection, spores undergo germination. During germination, the PT rapidly extends to form a long, linear tube, through which infectious cargo, called sporoplasm, is transported from the spore to the host cell18,19,20,21 (Fig. 1A). Once inside the host cell, E. intestinalis replicates within a parasitophorous vacuole (PV)5,22,23, also known as the sporophorous vesicle24. Similar to E. intestinalis, many other microsporidian species replicate within PVs, while other species replicate directly in the host cytosol25,26. The parasite replicates through a series of 4 stages: (1) the meront stage, an amorphous, multinucleate stage in which repeated nuclear divisions occur without cytokinesis5,22,23; (2) the sporont stage, in which cellularization occurs and the spore coat begins to form5,22,23; (3) the sporoblast stage, a post-replicative stage in which parasites start developing the specialized organelles found in mature spores, such as the PT5,22,23; and (4) the spore stage, which is the transmissible form of the parasite5,22,23. Spores are surrounded by a thick spore coat, which consists of a proteinaceous exospore layer, and a polysaccharide-rich endospore layer that contains chitin. The spore coat renders the spores resistant to the environment.
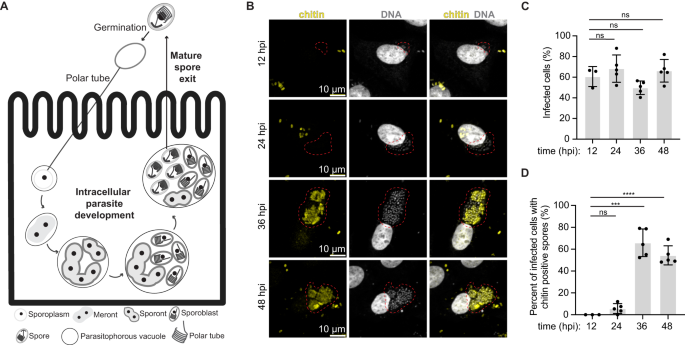
A Schematic of the E. intestinalis life cycle. B Micrographs of Vero cells infected with E. intestinalis and imaged at 12 hpi, 24 hpi, 36 hpi and 48 hpi. Cells are stained for DNA (DRAQ5, gray), chitin (calcofluor white, yellow). Infection clusters in cells are marked with a red dotted line. C Quantification of infected Vero cells at 12 hpi, 24 hpi, 36 hpi and 48 hpi. D Quantification of infected Vero cells that have chitin-positive infection clusters at 12 hpi, 24 hpi, 36 hpi and 48 hpi. Mean ± SD are from three biological replicates for 12 hpi and five biological replicates for 24 hpi, 36 hpi and 48 hpi. n = 100 cells per experiment. ****p < 0.0001, ***p < 0.001, ns, not significant (p > 0.05), calculated from an unpaired Student's t-test for (C, D). Source data for (C and D) are provided in the Source Data file.
Microsporidia are highly diverged from other eukaryotes. As an obligate intracellular parasite with the smallest known eukaryotic genome27, E. intestinalis lacks numerous pathways important for basic cellular metabolism, such as the electron transport chain27,28, and is highly dependent on the host for resources such as metabolites. For these reasons, microsporidia must establish an intracellular niche in order to avoid host detection and to promote its own replication, development and dissemination. Establishing an intracellular niche likely involves modulation of host cell physiology, in order to: (1) optimally position the PV within the host cell, (2) expand the PV membrane to accommodate the replicating parasites, and (3) siphon metabolites into the PV. Evidence from some Encephalitozoon spp. suggests that the PVs are in close proximity to the host mitochondrial network and nucleus29,30,31, which may facilitate the acquisition of ATP, lipids, and other molecules from the host. Interactions between mitochondria and PVs have been observed using 2D TEM as well as light microscopy, and a direct interaction between microsporidia and mitochondrial outer membrane proteins has also been proposed29. More recent data suggest that infection with some Encephalitozoon spp. can lead to Drp1-dependent mitochondrial fragmentation32.
The physical basis of the interactions within the E. intestinalis niche, and how the niche is established remain unclear. Moreover, our knowledge of how microsporidian parasites develop in a PV, and how organelles like the PT are built and organized during development remains rudimentary. To learn about these processes, 3-dimensional information on different parasite stages, and interactions between the parasite and the host in infected cells are needed. Advances in volume EM (vEM) techniques have enabled the reconstruction of large biological samples in 3D, at nanometer resolution, through a depth of several micrometers33. This makes vEM techniques, such as serial block-face scanning electron microscopy (SBF-SEM)34, excellent tools to study parasites and their interactions with hosts. vEM approaches have recently been used to investigate the ultrastructure of microsporidia and other eukaryotic parasites, both in isolation16 and during intracellular development35,36. To gain a better understanding of the E. intestinalis life cycle, intracellular parasite development, and host-cell interaction, we used SBF-SEM, as well as live-cell imaging. Our work reveals how parasite organelles develop throughout the E. intestinalis life cycle, the physical connections between the PV and host organelles, and the dynamics of parasite-mediated host mitochondrial remodeling. Using these data, we propose a model for how the PT and other organelles develop within the parasite.
Results
Correlative light and electron microscopy (CLEM) of host cells infected with E. intestinalis
To better understand the intracellular development and the host cell niche, we set out to obtain 3D snapshots of E. intestinalis-infected host cells using SBF-SEM34. To establish a timeline for the E. intestinalis life cycle in Vero cells and identify optimal infection time points for SBF-SEM experiments, we monitored infection using light microscopy. We used a fluorescent DNA dye (DRAQ5)37,38, which stains parasites of all developmental stages, and a fluorescent chitin dye (calcofluor white)39, which stains the spore coat, to detect parasites at more mature developmental stages. Meronts, sporonts and sporoblasts are expected to be DRAQ5-positive only, while spores, which have developed a chitin-rich endospore layer, are expected to be DRAQ5-positive and calcofluor white-positive. At all the time points analyzed, at least 50% of cells were infected (Fig. 1B, C). Very few chitin-positive infected cells are observed at early time points (12 hpi and 24 hpi), while more than 55% of infected cells are chitin positive at later time points (36 hpi and 48 hpi) (Fig. 1D). These data suggest that 12–24 hpi time points provide information on early parasite development, while 36–48 hpi time points provide information on more mature parasites. We therefore decided to image infected cells at 24 hpi and 48 hpi using SBF-SEM.
To prepare samples for SBF-SEM, we used a correlative light and volume EM (CLEM)40 workflow that we optimized for E. intestinalis-infected Vero cells (see "Methods"). The CLEM workflow was necessary in order to efficiently identify and image infected cells amidst neighboring uninfected cells. We first seeded Vero cells on a tissue culture dish with a gridded coverslip that has an identifiable pattern within each square. Following infection with E. intestinalis spores for either 24 h or 48 h, we used DIC light microscopy to identify infected cells of interest and record their location on the gridded coverslip. After fixation and embedding for EM, we used the grid location to relocate cells of interest as identified by light microscopy and examined them at an ultrastructural level using SBF-SEM (Fig. 2A, B). For each infection time point, we acquired two datasets, with each dataset containing a single infected cell (Fig. 2C, D; Supplementary Fig. 1A, C). In addition, we acquired three datasets for subcellular regions of infected cells at 48 hpi, with each region containing at least one parasitophorous vacuole (PV).
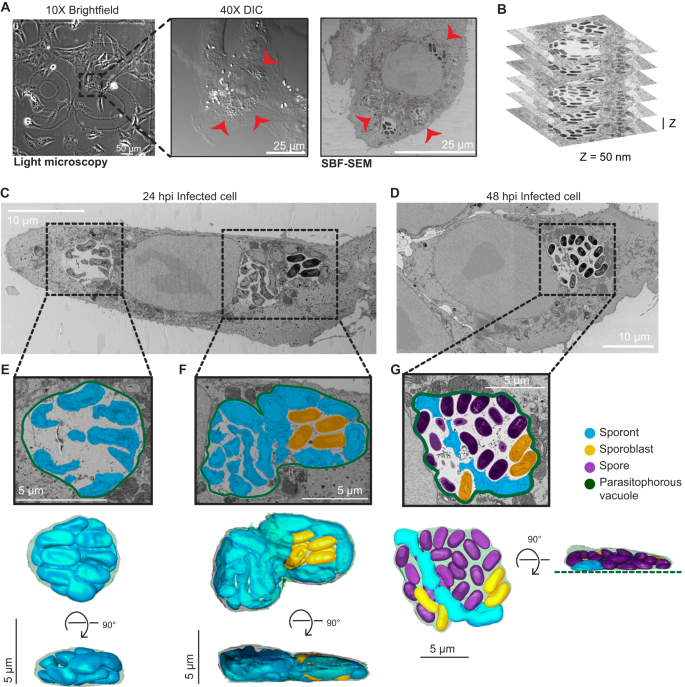
A Representative brightfield, differential interference contrast (DIC) and SBF-SEM micrographs of an infected cell at 24 hpi. The black dashed box indicates the infected cell in the corresponding higher magnification DIC micrograph (middle) and slice of SBF-SEM data (right). Red arrowheads indicate the parasitophorous vacuoles identified in a light microscopy image (middle) and in a slice of the SBF-SEM data (right). Datasets for two infected cells were acquired at 24 hpi, and two infected cells were acquired at 48 hpi. B Representative slices of SBF-SEM data, which were acquired serially with a Z-depth of 50 nm. C, D Representative slices from SBF-SEM datasets of infected cells at 24 hpi (C) and 48 hpi (D). Black dotted boxes indicate parasitophorous vacuoles that contain replicating E. intestinalis parasites at different stages of development. E–G Segmented parasitophorous vacuoles 24 hpi (E, F) and 48 hpi (G). Data for the vacuole in (G) is incomplete in our dataset, which did not capture the whole cell, as indicated by the dotted green line.
Across the seven datasets, we observed a total of 17 PVs: 11 PVs at 24 hpi and 6 PVs at 48 hpi (Supplementary Data 1). Overall, the PVs observed in Vero cells were flat, pancake-like structures (Fig. 2E, F; Supplementary Fig. 1B), consistent with the Vero cells themselves being relatively flat. We annotated, segmented and reconstructed PVs and parasites in 3D, to assess how parasites are packed within the PV. Consistent with previous TEM data, parasite development is not synchronous, and a single PV can contain parasites at various developmental stages5,22,23. As expected from our observations by light microscopy, in PVs at 24 hpi, predominantly earlier stages of parasite development are present. These are sporonts (60%) and sporoblasts (40%), which both lack a clear endospore layer (Fig. 2C, E, F; Supplementary Fig. 1A, B; Supplementary Fig. 2A). Although a proliferative, multinucleate plasmodial stage, or meront, is common in many microsporidian species, in E. intestinalis the meronts appear to be transient and replication appears to occur primarily during the sporont stage5,22,23. In line with this, we are unable to identify any meronts in our dataset. In PVs at 48 hpi, we observe more parasites in the later stages of development. The majority of parasites are spores (69%), with many fewer sporonts (7%) and sporoblasts (24%) (Fig. 2D, G; Supplementary Figs. 1C, D and 2A). In PVs containing only sporonts, these parasites lie very close to the PV membrane, where they are tightly packed and occupy ~80% of the PV volume (Fig. 2E; Supplementary Fig. 2B, C; Supplementary Movie 1). However, in larger PVs containing a mixture of parasites at different stages of development, large spaces are present between the developing parasites, and only ~40% of the PV is occupied by parasites (Fig. 2F, G; Supplementary Fig. 2B, C). When spaces are observed between parasites, they are often filled with vesicle-like structures that may be either secreted from the developing parasites or internalized from the host cytosol (Supplementary Fig. 2D, E). It is possible that similar to other intracellular parasites41,42,43, the uptake of vesicles from the host could be one mechanism of acquiring nutrients across the vacuole membrane. Aside from vesicles, we do not observe any discrete structures in inter-parasite spaces, which is in contrast to previous TEM data that shows the presence of a dense matrix between parasites5,22. This difference could be for several reasons, including differences in sources of our samples, or techniques used for imaging.
3D snapshots of E. intestinalis parasite development
To better understand how organelles are built and organized within the developing spore, we first classified parasites observed in our SBF-SEM datasets as sporonts, sporoblasts, or spores based on previous 2D TEM analyses of infected host cells5,22,23. We then segmented the parasite surface (plasma membrane or spore coat), in order to define the boundaries of each parasite, as well as any discernible internal organelles. From our segmentation analysis, we generated 3D reconstructions for parasites from each developmental stage.
Sporonts
All 17 of our reconstructed PVs contained at least 1 sporont, the earliest developmental stage observed in our datasets. We analyzed a total of 64 sporonts across the 17 PVs, from which we generated 3D reconstructions of 12. Our 3D reconstructions revealed that sporonts are irregular, flattened structures. The flattened appearance of sporonts may arise from the absence of most organelles at this stage, as well as from the flat shape of the PV. We observed sporonts as either single mononucleated cells (9 out of 64 cells; 9 nuclei), or more often as chains of semi-cellularized, multinucleated cells that occurred in multiples of 2 (55 out of 64 cells, containing a total of 162 nuclei) (Fig. 3A). Multinucleated sporont chains with two nuclei were observed most frequently (33 out of 64 cells; 66 nuclei), in addition to chains of four nuclei (20 out of 64 cells; 80 nuclei) and eight nuclei (2 out of 64 cells; 16 nuclei). The observation that the number of nuclei in sporont chains follows 2n for n = 0–3 suggests that genome replication and cytokinesis are synchronized within each chain. The parasite nucleus and a dense mesh of ER-like membrane were the only organelles discernable in our datasets at this stage of development (Fig. 3B; Supplementary Fig. 3A; Supplementary Movie 2). On average, sporonts are 2.3 µm3 in volume and ~3.5 µm in length along the anterior-posterior (A-P) axis (Fig. 3C; Supplementary Fig. 4A).
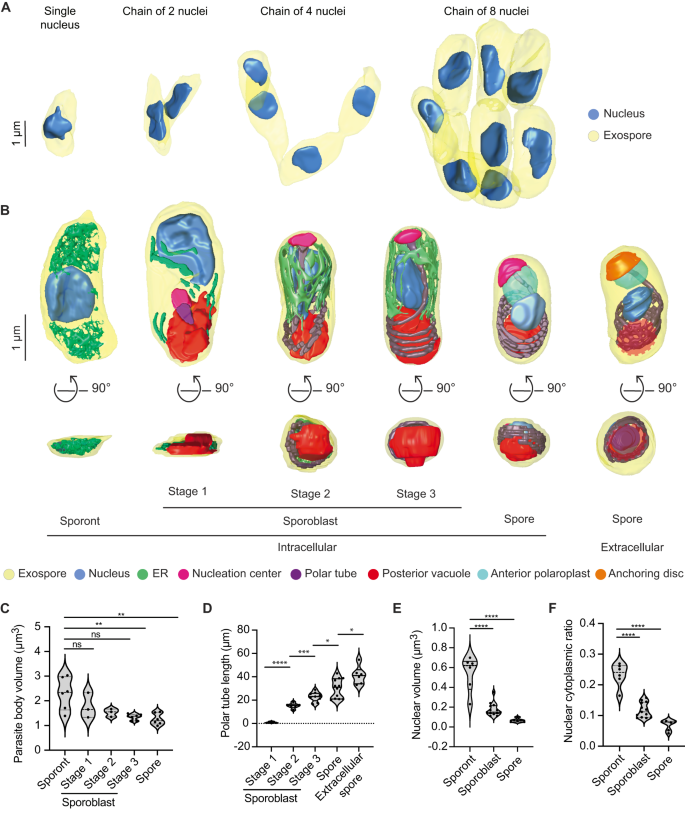
A 3D reconstructions of sporont chains containing 1, 2, 4 and 8 nuclei. B Representative 3D reconstructions of each E. intestinalis developmental stage. Posterior vacuole in extracellular spore indicated by dotted red circle. C Quantification of parasite volume at each developmental stage. D Quantification of polar tube length at each developmental stage. E Quantification of nuclear volume at each developmental stage. F Nuclear-cytoplasmic ratio calculated for each developmental stage. Violin plots show median and quartiles, *p < 0.05, **p < 0.01, ***p < 0.001, ****p < 0.0001; ns, not significant (p > 0.05), calculated from an unpaired Student's t-test for (C–F). Source data for (C–F) are provided in the Source Data file.
Sporoblasts
In addition to sporonts, six of the 17 PVs we reconstructed also contain sporoblasts, the next stage of development. In the sporoblast stage, cellularization has completed and the development of specialized parasite organelles begins. We analyzed 61 sporoblasts across all the PVs and generated 18 3D reconstructions. Following cell division, each sporoblast contains a single nucleus and is enveloped in its own plasma membrane (Fig. 3B; Supplementary Fig. 3B–D). At this stage of parasite development, the spore coat is not fully developed: the exospore layer is present, but the endospore layer is not discernable (Supplementary Fig. 3B–D). In addition to the parasite nucleus and a dense mesh of ER-like membrane, this is the first stage of development in which the PT and posterior vacuole are observed. Since the PT length increases as the parasite develops, up to a final length of ~41 µm in mature spores, we used the increasing length of the PT to classify sporoblasts into three stages. Stage 1: PT length less than 10 µm; Stage 2: PT length 10–15 µm; and Stage 3: PT length greater than 15 µm (Fig. 3B, D; Supplementary Movies 3–5). The average length of a sporoblast is 3 µm along the A-P axis across all three stages (Supplementary Fig. 4A). However the sporoblast volume changes from 1.8 µm3 in Stage 1 to 1.3 µm3 in Stage 3 (Fig. 3C). Across these stages, the developing PT is associated with an electron-dense organelle at one end, which we term the nucleation center due to a possible role in nucleating the growth of the PT (Fig. 3B; Supplementary Fig. 3B–D). Interestingly, previous work on Nosemoides vivieri also noted the presence of a morphologically similar structure, termed the sac polaire44, which is sometimes associated with the PT and Golgi-like vesicles. Strikingly, the overall shape of parasites transforms from flatter, elongated structures in Stage 1 to more 3-dimensional, bean-shaped structures by Stage 2, which may be related to the assembly of the PT.
Spores
Five of the six PVs from 48 hpi contain spores, the final intracellular stage of parasite development. We observed 140 spores across all PVs analyzed and generated 3D reconstructions of 12 spores. The spores are bean-shaped, ~2.5 µm in length along the A-P axis, and 1.26 µm3 in volume (Fig. 3C; Supplementary Fig. 4A). In addition to the organelles present in the earlier stages of parasite development, in the maturing spore, we also observe (1) clear presence of the endospore layer between the exospore and the plasma membrane, and (2) the lamellar polaroplast at the anterior end of the spore, where it surrounds the linear segment of the PT (Fig. 3B; Supplementary Fig. 3E; Supplementary Movie 6). However, compared to previous 3D spore reconstructions of the closely related Encephalitozoon hellem16, the anchoring disc is not visible in E. intestinalis spores observed in the PV at 48 hpi, and the endospore layer appears to be thinner. These observations, combined with the fact that the PVs still contain a significant percentage of parasites at earlier developmental stages, lead us to speculate that the spores within these PVs may not be fully mature. For comparison to fully mature, extracellular spores, we purified E. intestinalis spores after several days of infection in Vero cells and collected an SBF-SEM dataset. Overall, the appearance of purified spores is very similar to the spores observed within the PV. However, consistent with our hypothesis, the endospore layer is thicker in purified spores (~52 nm, vs. ~24 nm for spores within PVs), and the PT is clearly capped by the anchoring disc at the spore anterior (Fig. 3B; Supplementary Fig. 3F; Supplementary Movie 7). These observations suggest that the anchoring disk is one of the last organelles to develop in the spore. In addition, in purified spores, we do not observe a density corresponding to the texture of the density we have annotated as the nucleation center. This could be because (1) the nucleation center no longer remains once the PT is fully developed; (2) the nucleation center is not visible due to technical issues such as imperfect stain penetration; or (3) the nucleation center matures into the anchoring disc. As the shape of the anchoring disc and the nucleation center are strikingly similar, it is likely that the nucleation center matures into the anchoring disc, but that its composition changes, leading to a change in the appearance of the density.
In addition to the changes in parasite shape observed during development, we also found that parasite volume decreases from 2.29 µm3 in the sporonts to 1.3 µm3 in spores. Simultaneously, we observed a substantial decrease in nuclear volume, from 0.54 µm3 in sporonts to 0.06 µm3 in spores (Fig. 3E). The nuclear volume decreases more rapidly than that of the cytoplasm, such that the nuclear-cytoplasmic ratio (NC ratio) changes throughout development, from 0.19 in sporonts to 0.05 in spores (Fig. 3F). The NC ratio is usually constant in a given cell type and species45,46, and maintaining a constant NC ratio is tightly regulated both by biological factors47,48,49,50,51 and biophysical forces like osmotic pressure52. However, the NC ratio can vary considerably between cell types, and consequently, is known to change during cellular differentiation46. The reduction in NC ratio during E. intestinalis development could be the result of the spore becoming more metabolically dormant as it matures. Moreover, minimizing the NC ratio may allow for the nucleus to be small enough to be packaged and squeezed into the much narrower polar tube16, to be transported into a new host cell during infection.
Polar tube development and organization
Our 3D reconstructions provide new insights into how the PT organelle develops. Globally, the PT consists of a linear segment at the apical end, and multiple coils toward the posterior end of a mature spore (Fig. 4A). The question of how the PT develops has remained unanswered. Given that the length of the PT increases as the parasite matures, possible models of PT development include: (1) the coiled portion of the PT develops first, followed by assembly of the linear segment, (2) the linear segment of the PT develops first, followed by assembly of the coiled region or (3) preformed fragments of the PT coalesce at multiple junctures to form the PT44. With any of these models, it is unclear where the oldest and newest portions of the tube are. Here, our 3D models of the PT in different stages of development, provide some insights into the PT assembly process.
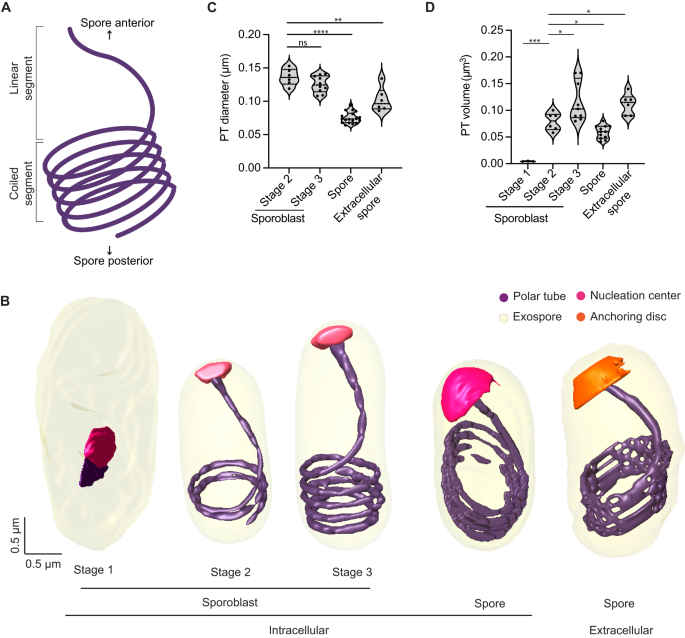
A Schematic illustrating the global organization of the polar tube, which contains a linear segment toward the anterior end of the spore and a coiled segment toward the posterior end. B Representative 3D reconstructions of the polar tube at each E. intestinalis developmental stage. C Quantification of polar tube diameter at each developmental stage. D Quantification of polar tube volume at each developmental stage. Violin plots show median and quartiles, *p < 0.05, **p < 0.01, ***p < 0.001, ****p < 0.0001; ns, not significant (p > 0.05), calculated from an unpaired Student's t-test for (C, D). Source data for (C and D) are provided in the Source Data file.
The first stage in which we can clearly identify the PT is Stage 1 sporoblasts, in which the PT is visible as a very short linear segment attached to the nucleation center (Fig. 4B). The nucleation center remains associated with the PT throughout its development, and it is possible that this electron-dense organelle serves as a site where new PT material is assembled. The sac polaire, observed previously in N. vivieri may be related to the nucleation center we observe. Although the texture of the density is different, the shape of the organelle is often similar. Previously, the sac polaire was often observed adjacent to Golgi-like vesicles, which were hypothesized to be the source of the PT44. In our SBF-SEM data, we are unable to identify the Golgi unambiguously. Focusing on the PT, we observed very few sporoblasts with short linear segments (Stage 1 sporoblasts; 4 out of 61), suggesting that this stage of PT growth is transient, and development rapidly proceeds to the next observed stage, in which the linear segment of the PT is substantially longer. As the PT length increases, the number of coils observed at the posterior end of the cell also increases. Stage 2 sporoblasts have 1–2 coils at the posterior end (24 out of 61), while more mature, Stage 3 sporoblasts have 3–4 coils (33 out of 61) (Fig. 4B). The appearance of PT coils in Stage 2 sporoblasts coincides with changes in the shape of the parasite. As the PT is fairly stiff on the micron-length scale, the coiling of the growing PT could serve as a mechanical scaffold, or ribcage, giving structure to the spore body. The PT coils may reduce the deformability of the parasites in later developmental stages, and provide their characteristic shape. We did not observe parasites in which the coiled portion of the PT exists without a linear segment, supporting a model of development in which the linear segment is formed first, followed by the progressive addition of coils as the parasites mature.
Similar to the PT organization in E. hellem spores and Anncaliia algerae16, the PT in E. intestinalis always forms a right-handed helix (Supplementary Fig. 4B). The E. intestinalis PT is anchored to the anterior end of the spore wall, slightly off-center with respect to the A-P axis (Supplementary Fig. 4C), as previously observed in E. hellem16. Although PT handedness is established early in parasite development (Stage 2 sporoblasts), the off-center anchoring of the PT with respect to the A-P axis is only observed in spores (Supplementary Fig. 4C). This change in PT positioning coincides with the development of (1) the endospore layer and (2) the lamellar polaroplast that snugly surrounds the linear segment of the PT. We also calculated the diameter and volume of the PT at different stages of parasite development (Fig. 4C, D). While PT length increases during development, its diameter decreases, with a 1.7-fold reduction in diameter from Stage 3 sporoblasts to spores (Fig. 4C). Given that the PT is composed of multiple layers53,54 (Supplementary Fig. 4D), it is unclear from the resolution of our SBF-SEM data whether these changes in PT diameter arise from changes in the proteins hypothesized to form the structural skeleton of the PT55,56,57,58, or from changes in the surrounding elements, such as membranes.
E. intestinalis niche in host cells
The PV membrane forms a boundary between the replicating parasites and the host cytoplasm. This boundary likely helps E. intestinalis avoid detection by host innate immune sensors while facilitating the acquisition of metabolites from the host. Our 3D reconstructions of infected cells allowed us to explore the physical interactions between PVs and host organelles. We segmented all discernible host cell organelles from the four infected cells described above, including the nucleus, mitochondria and ER (Fig. 5A, B; Supplementary Fig. 5A–F). To quantify interactions between the PV and host organelles, we scored two segmented organelles as forming a 'potential contact site' if the distance between them was equal to or less than 30 nm. We refer to these interactions as potential contact sites since the resolution of our SBF-SEM datasets does not allow us to ascertain if they physically interact (e.g., via protein-protein or protein-lipid interactions), or are merely in very close proximity. The largest number of potential contact sites (42% of all sites) are observed between the PV and the host mitochondria (Supplementary Fig. 5M). Potential contact sites can also be found between the ER and PV (33% of all sites). However, upon inspection of 3D-reconstructed cells at both 24 hpi and 48 hpi, it appears that the ER is not selectively recruited to the PVs, but rather is surrounding all organelles (Fig. 5A, B; Supplementary Fig. 5E, F). Potential contact sites are also observed between the nucleus and some PVs (25% of all sites). In infected cells with multiple PVs, at least one PV per cell abuts the host nucleus, but many do not (Fig. 5C, D; Supplementary Fig. 5G, H). When PVs are in close contact with the nucleus, we observe small indentations in the nuclear membrane (Supplementary Fig. 5L), consistent with previous 2D TEM images of Caco-2 cells infected with E. intestinalis31. Since PV proximity to the host nucleus is highly variable, we asked whether PVs containing specific parasite stages are more likely to form potential contact sites with the host nucleus. However, upon examining the composition of each PV, we did not find any correlation between the parasite developmental stage and the proximity of the PV to the nucleus (Fig. 5G, H; Supplementary Fig. 5K, M). The variability in the position of PVs relative to the host nucleus, coupled with the lack of any trend related to the parasite developmental stage, suggests that the proximity of the PVs to the host nucleus may simply reflect spatial constraints within the cell, rather than a specific interaction. In contrast to the positioning of PVs relative to the host nucleus, we observed that all PVs form potential contact sites with host mitochondria regardless of the parasite composition of the PV (Fig. 5E, F; Supplementary Fig. 5I, J, M).
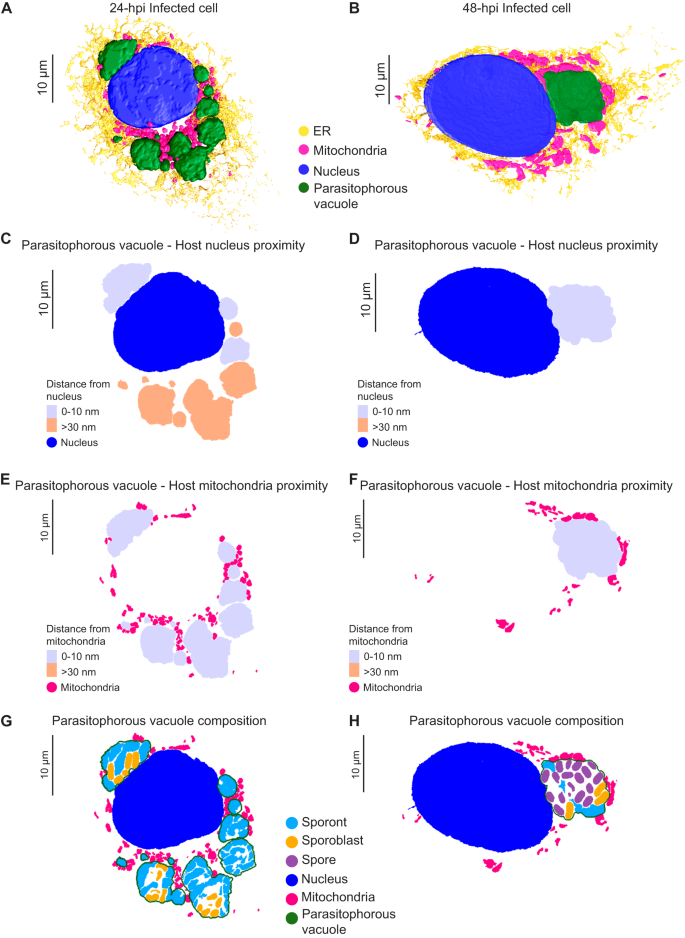
A, B 3D reconstructions of Vero cells infected with E. intestinalis at 24 hpi (A) or 48 hpi (B). C, D Distance maps showing the minimal distances of parasitophorous vacuoles from the host nucleus at 24 hpi (C) and 48 hpi (D). E, F Distance maps showing the minimal distances of parasitophorous vacuoles from the host mitochondria at 24 hpi (E) and 48 hpi (F). G, H Slices through 3D reconstructions of the intracellular niche showing parasitophorous vacuole composition at 24 hpi (G) and 48 hpi (H).
The consistency of the observed PV-mitochondria potential contact sites suggests that the interaction with host mitochondria is likely important for all parasite developmental stages, and unlike the ER and nucleus, host mitochondria appear to be selectively recruited to PVs, consistent with previous observations29,30,31. Given that microsporidia have highly reduced genomes, it is possible that these physical contacts with mitochondria allow the parasite to rapidly co-opt host metabolites for successful replication and development. In addition to physical proximity, our 3D reconstructions hinted at morphological changes in mitochondria in cells infected with E. intestinalis. To better understand these morphological changes, we segmented and compared the shape of individual mitochondria between a 24 hpi cell and an uninfected control. For each individual mitochondrion (see "Methods"), we then calculated an aspect ratio, which allowed us to assess if a mitochondrion was more elongated or more spherical. In uninfected cells the mitochondrial network is composed of consistently longer, linear, filamentous mitochondria (Fig. 6A, B; Supplementary Fig. 6A). In contrast, in infected cells, the mitochondrial network is composed of shorter and more spherical mitochondria (Fig. 6A, B; Supplementary Fig. 6A). Together, our data show that all PVs are in close contact with mitochondria, and the morphology of the mitochondrial network is altered, resulting in spheroid-shaped mitochondria59.
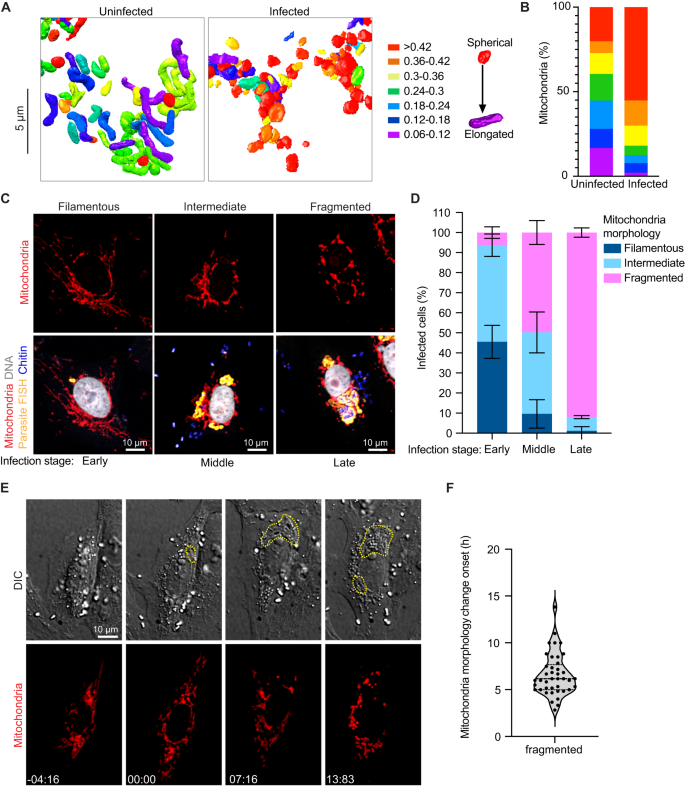
A 3D reconstructions of host mitochondria in an uninfected and infected cell, colored by aspect ratio, and B Quantification of mitochondrial aspect ratio from a given volume of an uninfected and infected cell. n ≥ 100 mitochondria from n = 2 uninfected and infected cells. C Vero cells infected with E. intestinalis, fixed and analyzed by E. intestinalis RNA FISH (yellow) and stained for DNA (DRAQ5, gray), chitin (calcofluor white, blue) and mitochondria (Tom70 antibody, red). Representative micrographs of infected cells and their host mitochondria morphology (top panel) and the corresponding E. intestinalis infection stage (bottom panel) are shown. D Quantification of mitochondrial morphology (filamentous, intermediate, or fragmented) in early, middle and late E. intestinalis infected Vero cells. Data are represented as mean ± SD from three biological replicates. n ≥ 100 cells per experiment. E Vero cells expressing DsRed targeted to the mitochondrial matrix were infected with E. intestinalis and imaged for 40 h. Differential interference contrast ...
Comments
Post a Comment